This week at the Royal Astronomical Society’s National Astronomy Meeting in the UK, physicists are challenging the evidence for the recent BICEP2 results regarding the inflation period of the Universe, announced just 90 days ago. New research is laying doubt upon the inclusion of inflation theory in the Standard Cosmological Model for understanding the forces of nature, the nature of elementary particles and the present state of the known Universe.
Back on March 17, 2014, it seemed the World was offered a glimpse of an ultimate order from eons ago … actually from the beginning of time. BICEP2, the single purpose machine at the South Pole delivered an image that after analysis, and subtraction of estimated background signal from the Milky Way, lead its researchers to conclude that they had found the earliest remnant from the birth of the Universe, a signature in ancient light that supported the theory of Inflation.
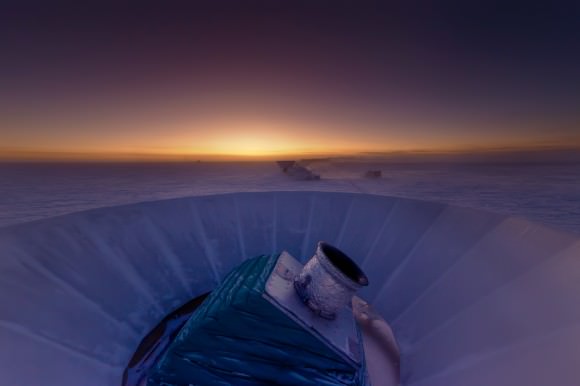
Thirty years ago, the Inflation theory was conceived by physicists Alan Guth and Andei Linde. Guth, Linde and others realized that a sudden expansion of the Universe at only 1/1000000000000000000000000000000000th of a second after the Big Bang could solve some puzzling mysteries of the Cosmos. Inflation could explain the uniformity of the cosmic background radiation. While images such as from the COBE satellite show a blotchy distribution of radiation, in actuality, these images accentuate extremely small variations in the background radiation, remnants from the Big Bang, variations on the order of 1/100,000th of the background level.
Note that the time of the Universe’s proposed Inflationary period immediately after the Big Bang would today permit light to travel only 1/1000000000000000th of the diameter of the Hydrogen atom. The Universe during this first moment of expansion was encapsulated in a volume far smaller than the a single atom.
Emotions ran very high when the BICEP2 team announced their findings on March 17 of this year. The inflation event that the background radiation data supported is described as a supercooling of the Cosmos however, there were physicists that simply remained cool and remained contrarians to the theory. Noted British Physicist Sir Roger Primrose was one who remained underwhelmed and stated that the incredible circular polarization of light that remained in the processed data from BICEP2 could be explained by the interaction of dust, light and magnetic fields in our own neighborhood, the Milky Way.
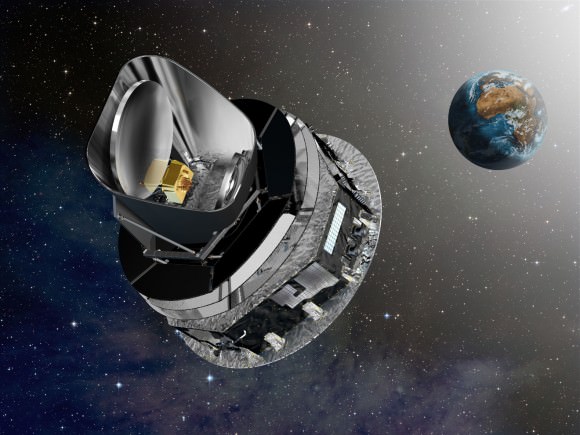
Now, new observations from another detector, one on the Planck Satellite orbiting the Earth, is revealing that the contribution of background radiation from local sources, the dust in the Milky Way, is appearing to have been under-estimated by the BICEP2 team. All the evidence is not yet laid out but the researchers are now showing reservations. At the same time, it does not dismiss the Inflation Theory. It means that more observations are needed and probably with greater sensitivity.
So why ask the question, are physicists constructing a Rube Goldberg device?
Our present understanding of the Universe stands upon what is called “the Standard Model” of Cosmology. At the Royal Astronomical Society meeting this week, the discussions underfoot could be revealing a Standard Model possibly in a state of collapse or simply needing new gadgets and mechanisms to remain the best theory of everything.
Also this week, new data further supports the discovery of the Higg’s Boson by the Large Hadron Collider in 2012, the elementary particle whose existence explains the mass of fundamental particles in nature and that supports the existence of the Higgs Field vital to robustness of the Standard Model. However, the Higgs related data is also revealing that if the inflationary period of the Universe did take place, then if taken with the Standard Model, one can conclude that the Universe should have collapsed upon itself and our very existence today would not be possible.
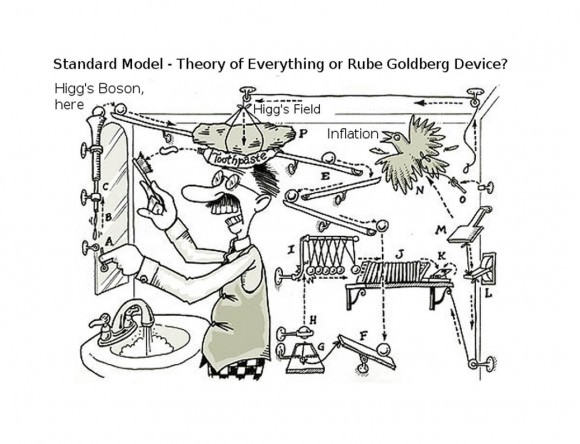
Dr. Brian Green, a researcher in the field of Super String Theory and M-Theory and others such as Dr. Stephen Hawking, are quick to state that the Standard Model is an intermediary step towards a Grand Unified Theory of everything, the Universe. The contortion of the Standard Model, into a sort of Rube Goldberg device can be explained by the undaunting accumulation of more acute and diverse observations at cosmic and quantum scales.
Discussions at the Royal Astronomical Society meeting are laying more doubts upon the inflation theory which just 90 days ago appeared so well supported by BICEP2 – data derived by truly remarkable cutting edge electronics developed by NASA and researchers at the California Institute of Technology. The trials and tribulations of these great theories to explain everything harken back to the period just prior to Einstein’s Miracle Year, 1905. Fragmented theories explaining separately the forces of nature were present but also the accumulation of observational data had reached a flash point.
Today, observations from BICEP2, NASA and ESA great space observatories, sensitive instruments buried miles underground and carefully contrived quantum experiments in laboratories are making the Standard Model more stressed in explaining everything, the same model so well supported by the Higg’s Boson discovery just two years ago. Cosmologists concede that we may never have a complete, proven theory of everything, one that is elegant; however, the challenges upon the Standard Model and inflation will surely embolden younger theorists to double the efforts in other theoretical work.
For further reading:
RAS NAM press release: Should the Higgs Boson Have Caused our Universe To Collapse?
We’ve Discovered Inflation!: Now What?
Cosmologists Cast Doubt on Inflation Evidence
Are the BICEP2 Results Invalid? Probably Not