[/caption]
Space is far from empty. The Solar System can be viewed as a “bubble” of solar matter – filled with particles emitted by the Sun as the solar wind – extending well beyond the orbit of Pluto. The solar wind velocity is supersonic for most of this distance (exceeding a million miles per hour), but the point at which it begins to interact with the interstellar medium (ISM), the solar wind drops to subsonic velocities, creating a region of compression known as the termination shock. After 26 years of flight, the Voyager 1 deep space probe entered this bizarre, turbulent region of space, where solar particles build up and magnetic fields become twisted. Now a new mission has been designed to watch this region of space from afar to begin to understand the boundary of our solar system, where violent turbulence rules and high-energy atoms are generated…
In 2004, Voyager 1 hit it and in 2006, Voyager 2 hit it. The first probe flew through the termination shock at around 94 AU (8 billion miles away); the second measured it at only 76 AU (7 billion miles). This result alone suggests that the termination shock may be irregularly shaped and/or variable depending on solar activity. Before the Voyager missions, the termination shock was theorized, but there was little observational evidence until the two veteran probes traversed the region. The termination shock is of paramount importance to understanding the nature of the outer reaches of the solar system as, counter-intuitively, the Sun’s activity increases, the region beyond the termination shock (the heliosheath) becomes more efficient at blocking deadly cosmic rays. During solar minimum, it becomes less efficient at blocking cosmic rays.
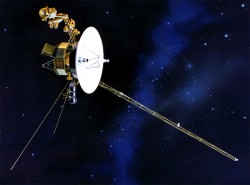
In an effort to map the location and characteristics of the termination shock and heliosheath beyond, NASA scientists are preparing the Interstellar Boundary Explorer (IBEX) for launch in October. IBEX is part of NASA’s Small Explorer program (SMEX), where inexpensive, small probes are used to efficiently observe particular cosmic phenomena. IBEX will be orbiting beyond the influence of the Earth’s magnetic field (the magnetosphere) at a 200,000 mile distance from the Earth. This is because the phenomenon IBEX will be observing can be generated by our own magnetic field. So what will IBEX be measuring? To understand the interaction between solar wind ions and the interstellar medium, IBEX will use two sensors to detect energetic neutral atoms (ENAs) being blasted from the outermost reaches of the solar system.
How are ENAs generated and how are they a measurement of the interaction between the heliosphere and the ISM? Out there in the ISM exists neutral atoms and ions. As the solar system passes through interstellar space, the strong magnetic field generated around the heliosphere deflects the charged ions, pushing them out of the way. However, slow-moving neutral atoms are not affected by the magnetic field and penetrate deep into the heliosheath. When this happens, these neutral atoms from the ISM interact with energetic protons (which do have charge) rapidly spiralling along the magnetic field embedded in the solar wind. When this interaction occurs (known as charge exchange), an electron is stripped from the ISM atom and attracted to the energetic solar wind proton, thus making it neutral. When this exchange occurs, an energetic hydrogen atom (electron and proton) is ejected. An ENA is born.
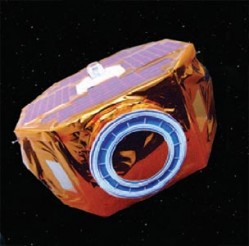
Now, this is where the clever bit comes in. As mentioned before, neutral atoms do not “feel” magnetic fields, so when ENAs are created they are ejected in a straight line. Some of these atoms will be directed toward the Earth. IBEX will then measure these ENAs and work out where they came from. As they will have travelled directly to IBEX, the location of the termination shock may be deduced. Over a period of time, IBEX will be able to build up a picture of the locations of these atomic interactions and relate them the characteristics of the boundary of our Solar System.
But the best thing is, we won’t need to send a probe into deep space and wait for decades before it traverses the boundary layer, we will be able to make these measurements from Earth orbit. Such an exciting mission. Roll on the Pegasus rocket launch October 5th, 2008!
Source: Physorg.com