Everyone knows just how fun magnets can be. As a child, who among us didn’t love to see if we could make our silverware stick together? And how about those little magnetic rocks that we could arrange to form just about any shape because they stuck together? Well, magnetism is not just an endless source of fun or good for scientific experiments; it’s also one of basic physical laws upon which the universe is based.
The attraction known as magnetism occurs when a magnetic field is present, which is a field of force produced by a magnetic object or particle. It can also be produced by a changing electric field and is detected by the force it exerts on other magnetic materials. Hence why the area of study dealing with magnets is known as electromagnetism.
Definition:
Magnetic fields can be defined in a number of ways, depending on the context. However, in general terms, it is an invisible field that exerts magnetic force on substances which are sensitive to magnetism. Magnets also exert forces and torques on each other through the magnetic fields they create.
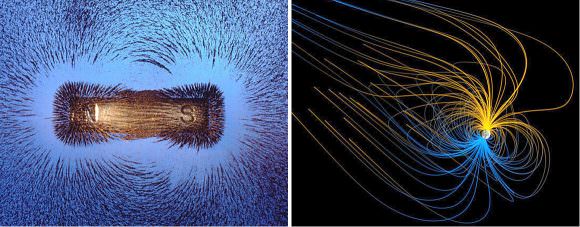
They can be generated within the vicinity of a magnet, by an electric current, or a changing electrical field. They are dipolar in nature, which means that they have both a north and south magnetic pole. The Standard International (SI) unit used to measure magnetic fields is the Tesla, while smaller magnetic fields are measured in terms of Gauss (1 Tesla = 10,000 Guass).
Mathematically, a magnetic field is defined in terms of the amount of force it exerted on a moving charge. The measurement of this force is consistent with the Lorentz Force Law, which can be expressed as F= qvB, where F is the magnetic force, q is the charge, v is the velocity, and the magnetic field is B. This relationship is a vector product, where F is perpendicular (->) to all other values.
Field Lines:
Magnetic fields may be represented by continuous lines of force (or magnetic flux) that emerge from north-seeking magnetic poles and enter south-seeking poles. The density of the lines indicate the magnitude of the field, being more concentrated at the poles (where the field is strong) and fanning out and weakening the farther they get from the poles.
A uniform magnetic field is represented by equally-spaced, parallel straight lines. These lines are continuous, forming closed loops that run from north to south, and looping around again. The direction of the magnetic field at any point is parallel to the direction of nearby field lines, and the local density of field lines can be made proportional to its strength.
Magnetic field lines resemble a fluid flow, in that they are streamlined and continuous, and more (or fewer lines) appear depending on how closely a field is observed. Field lines are useful as a representation of magnetic fields, allowing for many laws of magnetism (and electromagnetism) to be simplified and expressed in mathematical terms.
History of Study:
The study of magnetic fields began in 1269 when French scholar Petrus Peregrinus de Maricourt mapped out the magnetic field of a spherical magnet using iron needles. The places where these lines crossed he named “poles” (in reference to Earth’s poles), which he would go on to claim that all magnets possessed.
During the 16th century, English physicist and natural philosopher William Gilbert of Colchester replicated Peregrinus’ experiment. In 1600, he published his findings in a treaties (De Magnete) in which he stated that the Earth is a magnet. His work was intrinsic to establishing magnetism as a science.
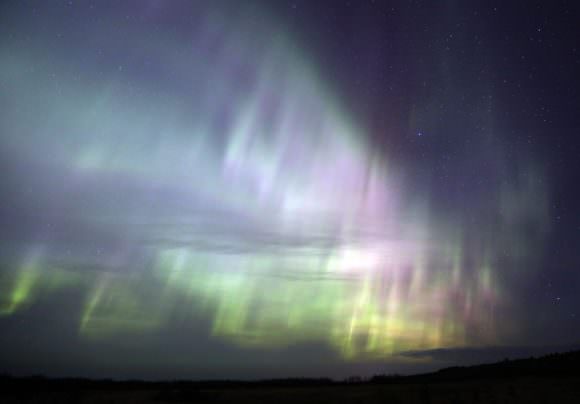
In 1750, English clergyman and philosopher John Michell stated that magnetic poles attract and repel each other. The force with which they do this, he observed, is inversely proportional to the square of the distance, otherwise known as the inverse square law.
In 1785, French physicist Charles-Augustin de Coulomb experimentally verified Earths’ magnetic field. This was followed by 19th century French mathematician and geometer Simeon Denis Poisson created the first model of the magnetic field, which he presented in 1824.
By the 19th century, further revelations refined and challenged previously-held notions. For example, in 1819, Danish physicist and chemist Hans Christian Orsted discovered that an electric current creates a magnetic field around it. In 1825, André-Marie Ampère proposed a model of magnetism where this force was due to perpetually flowing loops of current, instead of the dipoles of magnetic charge.
In 1831, English scientist Michael Faraday showed that a changing magnetic field generates an encircling electric field. In effect, he discovered electromagnetic induction, which was characterized by Faraday’s law of induction (aka. Faraday’s Law).
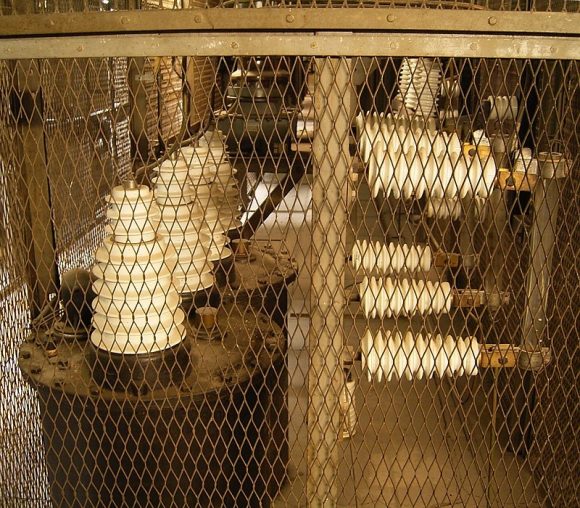
Between 1861 and 1865, Scottish scientist James Clerk Maxwell published his theories on electricity and magnetism – known as the Maxwell’s Equations. These equations not only pointed to the interrelationship between electricity and magnetism, but showed how light itself is an electromagnetic wave.
The field of electrodynamics was extended further during the late 19th and 20th centuries. For instance, Albert Einstein (who proposed the Law of Special Relativity in 1905), showed that electric and magnetic fields are part of the same phenomena viewed from different reference frames. The emergence of quantum mechanics also led to the development of quantum electrodynamics (QED).
Examples:
A classic example of a magnetic field is the field created by an iron magnet. As previously mentioned, the magnetic field can be illustrated by surrounding it with iron filings, which will be attracted to its field lines and form in a looping formation around the poles.
Larger examples of magnetic fields include the Earth’s magnetic field, which resembles the field produced by a simple bar magnet. This field is believed to be the result of movement in the Earth’s core, which is divided between a solid inner core and molten outer core which rotates in the opposite direction of Earth. This creates a dynamo effect, which is believed to power Earth’s magnetic field (aka. magnetosphere).
Other celestial bodies have been shown to have magnetic fields of their own. This includes the gas and ice giants of the Solar System – Jupiter, Saturn, Uranus and Neptune. Jupiter’s magnetic field is 14 times as powerful as that of Earth, making it the strongest magnetic field of any planetary body. Jupiter’s moon Ganymede also has a magnetic field, and is the only moon in the Solar System known to have one.
Mars is believed to have once had a magnetic field similar to Earth’s, which was also the result of a dynamo effect in its interior. However, due to either a massive collision, or rapid cooling in its interior, Mars lost its magnetic field billions of years ago. It is because of this that Mars is believed to have lost most of its atmosphere, and the ability to maintain liquid water on its surface.
When it comes down to it, electromagnetism is a fundamental part of our Universe, right up there with nuclear forces and gravity. Understanding how it works, and where magnetic fields occur, is not only key to understanding how the Universe came to be, but may also help us to find life beyond Earth someday.
We have written many articles about the magnetic field for Universe Today. Here’s What is Earth’s Magnetic Field, Is Earth’s Magnetic Field Ready to Flip?, How Do Magnets Work?, Mapping The Milky Way’s Magnetic Fields – The Faraday Sky, Magnetic Fields in Spiral Galaxies – Explained at Last?, Astronomy Without A Telescope – Cosmic Magnetic Fields.
If you’d like more info on Earth’s magnetic field, check out NASA’s Solar System Exploration Guide on Earth. And here’s a link to NASA’s Earth Observatory.
We’ve also recorded an episode of Astronomy Cast all about planet Earth. Listen here, Episode 51: Earth.
Sources: