Astronomers have been watching a nearby pulsar with a strange halo around it. That pulsar might answer a question that’s puzzled astronomers for some time. The pulsar is named Geminga, and it’s one of the nearest pulsars to Earth, about 800 light years away in the constellation Gemini. Not only is it close to Earth, but Geminga is also very bright in gamma rays.
Continue reading “Halo Around a Pulsar could Explain Why We See Antimatter Coming from Space”Dark Matter Could Be A Source of Gamma Rays Coming from the Center of the Milky Way
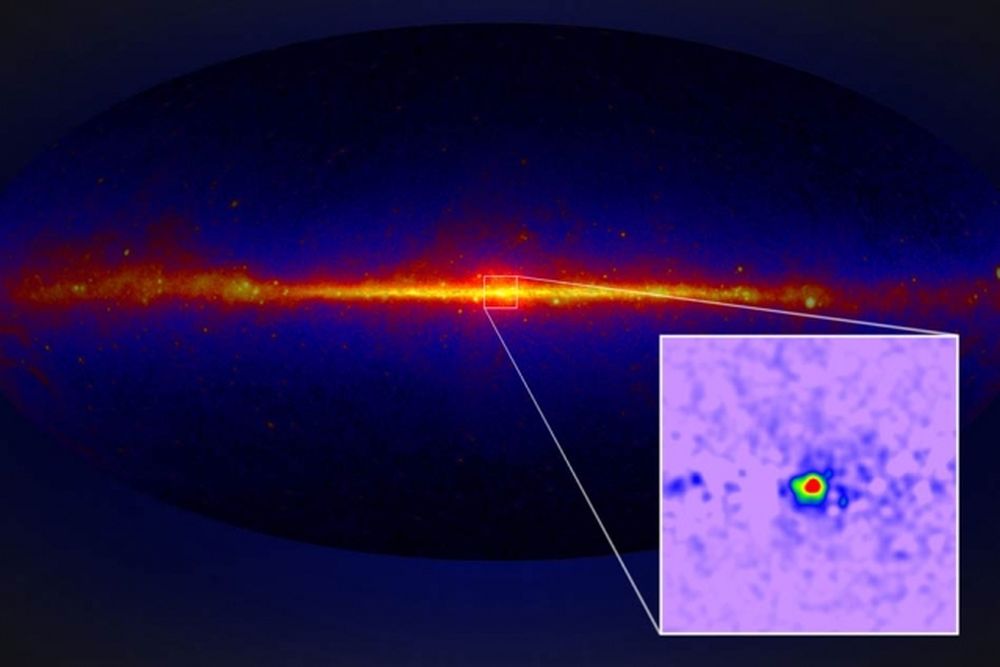
There’s a lot of mysterious goings-on at the center of the Milky Way. The supermassive black hole that resides there is chief among them. But there’s another intriguing puzzle there: an unexpected spherical region of intense gamma ray emissions.
A new study suggests that dark matter could be behind those emissions.
Continue reading “Dark Matter Could Be A Source of Gamma Rays Coming from the Center of the Milky Way”When it Comes to Gamma Radiation, the Moon is Actually Brighter Than the Sun

The eerie, hellish glow coming from the Moon may seem unreal in this image, since it’s invisible to our eyes. But instruments that detect gamma rays tell us it’s real. More than just a grainy, red picture, it’s a vivid reminder that there’s more going on than meets human eyes.
It’s also a reminder that any humans that visit the Moon need to be protected from this high-energy radiation.
Continue reading “When it Comes to Gamma Radiation, the Moon is Actually Brighter Than the Sun”Gamma Ray Telescopes could Detect Starships Powered by Black Hole

In the course of looking for possible signs of Extra-Terrestrial Intelligence (ETI), scientists have had to do some really outside-of-the-box thinking. Since it is a foregone conclusion that many ETIs would be older and more technologically advanced than humanity, those engaged in the Search for Extra-Terrestrial Intelligence (SETI) have to consider what a more advanced species would be doing.
A particularly radical idea that has been suggested is that spacefaring civilizations could harness radiation emitted from black holes (Hawking radiation) to generate power. Building on this, Louis Crane – a mathematician from Kansas State University (KSU) – recently authored a study that suggests how surveys using gamma telescopes could find evidence of spacecraft powered by tiny artificial black holes.
Continue reading “Gamma Ray Telescopes could Detect Starships Powered by Black Hole”Every Time Lightning Strikes, Matter-Antimatter Annihilation Happens too

Lighting has always been a source of awe and mystery for us lowly mortals. In ancient times, people associated it with Gods like Zeus and Thor, the fathers of the Greek and Norse pantheons. With the birth of modern science and meteorology, lighting is no longer considered the province of the divine. However, this does not mean that the sense of mystery it carries has diminished one bit.
For example, scientists have found that lightning occurs in the atmospheres of other planets, like the gas giant Jupiter (appropriately!) and the hellish world of Venus. And according to a recent study from Kyoto University, gamma rays caused by lighting interact with air molecules, regularly producing radioisotopes and even positrons – the antimatter version of electrons.
The study, titled “Photonuclear Reactions Triggered by Lightning Discharge“, recently appeared in the scientific journal Nature. The study was led by Teruaki Enoto, a researcher from The Hakubi Center for Advanced Research at Kyoto University, and included members from the University of Tokyo, Hokkaido University, Nagoya University, the RIKEN Nishina Center, the MAXI Team, and the Japan Atomic Energy Agency.
For some time, physicists have been aware that small bursts of high-energy gamma rays can be produced by lightning storms – what are known as “terrestrial gamma-ray flashes”. They are believed to be the result of static electrical fields accelerating electrons, which are then slowed by the atmosphere. This phenomenon was first discovered by space-based observatories, and rays of up to 100,000 electron volts (100 MeV) have been observed.
Given the energy levels involved, the Japanese research team sought to examine how these bursts of gamma rays interact with air molecules. As Teruaki Enoto from Kyoto University, who leads the project, explained in a Kyoto University press release:
“We already knew that thunderclouds and lightning emit gamma rays, and hypothesized that they would react in some way with the nuclei of environmental elements in the atmosphere. In winter, Japan’s western coastal area is ideal for observing powerful lightning and thunderstorms. So, in 2015 we started building a series of small gamma-ray detectors, and placed them in various locations along the coast.”
Unfortunately, the team ran into funding problems along the way. As Enoto explained, they decided to reach out to the general public and established a crowdfunding campaign to fund their work. “We set up a crowdfunding campaign through the ‘academist’ site,” he said, “in which we explained our scientific method and aims for the project. Thanks to everybody’s support, we were able to make far more than our original funding goal.”
Thanks to the success of their campaign, the team built and installed particle detectors across the northwest coast of Honshu. In February of 2017, they installed four more detectors in Kashiwazaki city, which is a few hundred meters away from the neighboring town of Niigata. Immediately after the detectors were installed, a lightning strike took place in Niigata, and the team was able to study it.
What they found was something entirely new and unexpected. After analyzing the data, the team detected three distinct gamma-ray bursts of varying duration. The first was less than a millisecond long, the second was gamma ray-afterglow that took several milliseconds to decay, and the last was a prolonged emission lasting about one minute. As Enoto explained:
“We could tell that the first burst was from the lightning strike. Through our analysis and calculations, we eventually determined the origins of the second and third emissions as well.”
They determined that the second afterglow was caused by the lightning reacting with nitrogen in the atmosphere. Essentially, gamma rays are capable of causing nitrogen molecules to lose a neutron, and it was the reabsorption of these neutrons by other atmospheric particles that produced the gamma-ray afterglow. The final, prolonged emission was the result of unstable nitrogen atoms breaking down.
It was here that things really got interesting. As the unstable nitrogen broke down, it released positrons that then collided with electrons, causing matter-antimatter annihilations that released more gamma rays. As Enoto explained, this demonstrated, for the first time that antimatter is something that can occur in nature due to common mechanisms.
“We have this idea that antimatter is something that only exists in science fiction,” he said. “Who knew that it could be passing right above our heads on a stormy day? And we know all this thanks to our supporters who joined us through ‘academist’. We are truly grateful to all.”
If these results are indeed correct, than antimatter is not the extremely rare substance that we tend to think it is. In addition, the study could present new opportunities for high-energy physics and antimatter research. All of this research could also lead to the development of new or refined techniques for creating it.
Looking ahead, Enoto and his team hopes to conduct more research using the ten detectors they still have operating along the coast of Japan. They also hope to continue involving the public with their research, a process that goes far beyond crowdfunding and includes the efforts of citizen scientists to help process and interpret data.
Further Reading: University of Kyoto, Nature, NASA Goddard Media Studios
A New Prototype Telescope Proves Itself Worthy
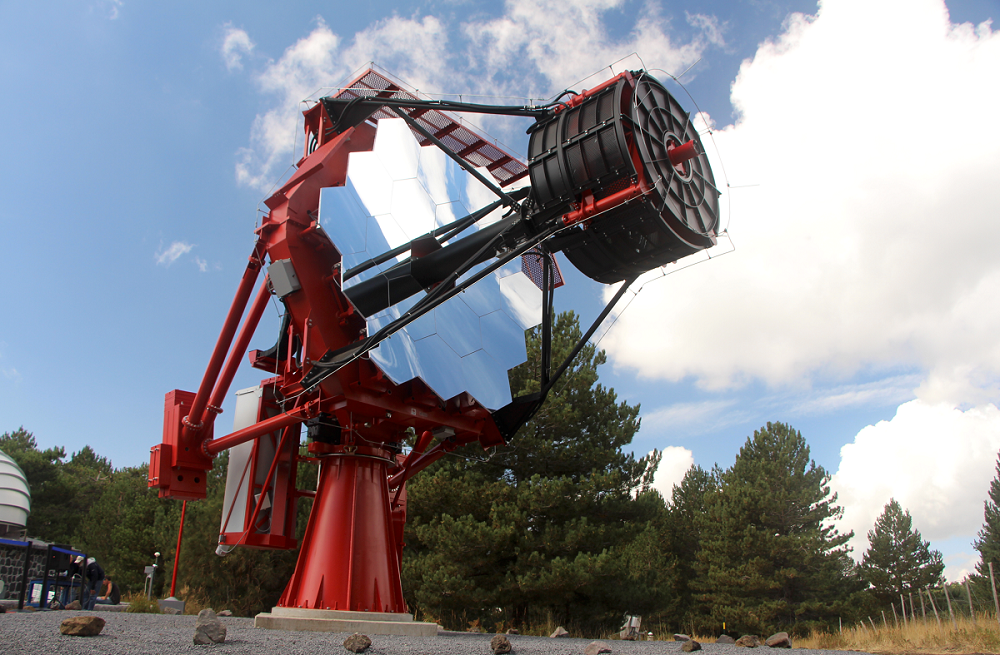
In 2013, the Cherenkov Telescope Array (CTA) was established with the intention of building the world’s largest and most sensitive high-energy gamma ray observatory. Consisting of over 1350 scientists from 210 research institutes in 32 countries, this observatory will use 100 telescopes across the northern and southern hemispheres to explore the high-energy Universe.
Key to their efforts is a prototype dual-mirror Schwarzschild-Couder telescope, known as the Astrofisica con Specchi a Tecnologia Replicante Italiana (ASTRI). Since it was first created in 2014, this prototype has been undergoing tests at the Serra La Nave Observing Station on Mount Etna, Sicily. And as of October of 2016, it passed its most important test to date, demonstrating a constant point-spread function across its full field of view.
The ASTRI telescope is essentially a revolutionary kind of Imaging Atmospheric Cherenkov Telescope (IACT). These ground-based telescopes are used by astronomers to detect cosmic high-energy gamma rays. These rays are produced by the most energetic objects in the universe (i.e. pulsars, supernovae, regions around black holes), and are only detectable because of the Cherenkov Effect, which they undergo once they pass into our atmosphere.
This effect occurs when particles of light achieve speeds greater than the phase velocity of light in their particular medium. In this case, the effect is produced when light particles pass from the vacuum of space into our atmosphere, temporarily exceeding the speed of light in air and producing a glow in the blue to UV range. In the case of very-high-energy gamma rays, indirect observations of this Cherenkov radiation is the only way to detect them.
Typically, Cherenkov telescopes use a mirror to collect light and focus it on a camera. The ASTRI telescope is something quite different, in that it is based on the Schwarzschild-Couder model. As Giovanni Pareschi, an astronomer at the INAF-Brera Astronomical Observatory and the principal investigator of the ASTRI project, told Universe Today via email:
“The ASTRI telescope for the first time is based on a two mirror imaging configuration (while in general Cherenkov telescopes work with in single mirror configuration, i.e. just a big primary mirror with the camera put in the Newtonian focus and a f-number close to 1). ASTRI is a prototype of the telescopes of the Small Size Telescope sub-array of the CTA Observatory. The sub-array is devoted to detect the gamma rays with the highest energy (up to 100 Tev). In order to properly work, the sub-array has to be based on a large number of telescopes (70 units) with a distance from each other of a 250 m distributed and with a large field (10 deg x 10 deg) of view with a constant angular resolution of a few arc minutes across the field of view.
This idea for such a telescope was first proposed in 1905 by German astronomer Karl Schwarzschild, but remained dormant for almost a century since it was deemed too difficult and too expensive to construct. It was not until 2007 that it was considered as a viable means for creating a new type of IACT. And in 2014, the INAF-Brera Astronomical Observatory commissioned the first of its kind to be built.
“[W]e have for the first time adopted a two reflection design based on the Schwarzschild-Couder configuration never realized before (also for telescopes operating in the visible band),” added Pareschi. “This configuration allows us to optimize the angular resolution across the field of view and to use focal plane cameras of small dimensions (thanks to this property, we could use new solid-state technology based Silicon photomultiplier sensors instead of the “old” classical photomultiplier tubes used so far in Cherenkov astronomy).”
These advantages, and the advances they allow for, will make ASTRI telescope approximately ten times more sensitive than current instruments. And with this latest test – which demonstrating a constant point-spread function of a few arc minutes over a large field of view of 10 degrees – the team behind it now has proof that it will work. As Pareschi explained:
“The test demonstrated for the first time that a telescope based on the Schwarzschild-Couder configuration correctly works and that a two-mirror configuration can be adopted for making Cherenkov telescopes for gamma ray astronomy. In addition, the ASTRI prototype has been completely characterized and validated from the opto-mechanical point of view, demonstrating that we can now proceed with the construction of the Small-Sized Telescopes (SSTs) of the array based on the ASTRI design.”
With this important test complete, the INAF-Brera team hopes to spend the next few months prepping the telescope. This will include mounting the Cherenkov camera onto the prototype and testing its gamma-ray performance. Then they will start to produce the first set of ASTRI telescopes to create a mini-array, which will serve as a precursor to the planned CTA sub-array that is scheduled to be built in Chile.
Once the camera is tested and mounted, the ASTRI team will conduct their first observations of gamma-rays at very high energies. These observations will allow scientists to determine the direction of gamma-ray photons that are the result of celestial sources, such as neutron stars, pulsars, supernovae, and black holes, tracing them back to their respective sources.
And with the planned construction of 100 SSTs to be spread out over the northern and southern hemispheres, the CTA array will outnumber all other telescopes in the world. The wide coverage and large number of these telescopes, spread over a wide area, will improve astronomers chances of detecting very high-energy gamma rays as they pass into our atmosphere.
Further Reading: CTA
Moonlight Is a Many-Splendored Thing
“By the Light of the Silvery Moon” goes the song. But the color and appearance of the Moon depends upon the particular set of eyes we use to see it. Human vision is restricted to a narrow slice of the electromagnetic spectrum called visible light.
With colors ranging from sumptuous violet to blazing red and everything in between, the diversity of the visible spectrum provides enough hues for any crayon color a child might imagine. But as expansive as the visual world’s palette is, it’s not nearly enough to please astronomers’ retinal appetites.
Since the discovery of infrared light by William Herschel in 1800 we’ve been unshuttering one electromagnetic window after another. We build telescopes, great parabolic dishes and other specialized instruments to extend the range of human sight. Not even the atmosphere gets in our way. It allows only visible light, a small amount of infrared and ultraviolet and selective slices of the radio spectrum to pass through to the ground. X-rays, gamma rays and much else is absorbed and completely invisible.
To peer into these rarified realms, we’ve lofting air balloons and then rockets and telescopes into orbit or simply dreamed up the appropriate instrument to detect them. Karl Jansky’s homebuilt radio telescope cupped the first radio waves from the Milky Way in the early 1930s; by the 1940s sounding rockets shot to the edge of space detected the high-frequency sizzle of X-rays. Each color of light, even the invisible “colors”, show us a new face on a familiar astronomical object or reveal things otherwise invisible to our eyes.
So what new things can we learn about the Moon with our contemporary color vision?
Radio: Made using NRAO’s 140-ft telescope in Green Bank, West Virginia. Blues and greens represent colder areas of the moon and reds are warmer regions. The left half of Moon was facing the Sun at the time of the observation. The sunlit Moon appear brighter than the shadowed portion because it radiates more heat (infrared light) and radio waves.
Submillimeter: Taken using the SCUBA camera on the James Clerk Maxwell Telescope in Hawaii. Submillimeter radiation lies between far infrared and microwaves. The Moon appears brighter on one side because it’s being heated by Sun in that direction. The glow comes from submillimeter light radiated by the Moon itself. No matter the phase in visual light, both the submillimeter and radio images always appear full because the Moon radiates at least some light at these wavelengths whether the Sun strikes it or not.
Mid-infrared: This image of the Full Moon was taken by the Spirit-III instrument on the Midcourse Space Experiment (MSX) at totality during a 1996 lunar eclipse. Once again, we see the Moon emitting light with the brightest areas the warmest and coolest regions darkest. Many craters look like bright dots speckling the lunar disk, but the most prominent is brilliant Tycho near the bottom. Research shows that young, rock-rich surfaces, such as recent impact craters, should heat up and glow more brightly in infrared than older, dust-covered regions and craters. Tycho is one of the Moon’s youngest craters with an age of just 109 million years.
Near-infrared: This color-coded picture was snapped just beyond the visible deep red by NASA’s Galileo spacecraft during its 1992 Earth-Moon flyby en route to Jupiter. It shows absorptions due to different minerals in the Moon’s crust. Blue areas indicate areas richer in iron-bearing silicate materials that contain the minerals pyroxene and olivine. Yellow indicates less absorption due to different mineral mixes.
Visible light: Unlike the other wavelengths we’ve explored so far, we see the Moon not by the light it radiates but by the light it reflects from the Sun.
The iron-rich composition of the lavas that formed the lunar “seas” give them a darker color compared to the ancient lunar highlands, which are composed mostly of a lighter volcanic rock called anorthosite.
Ultraviolet: Similar to the view in visible light but with a lower resolution. The brightest areas probably correspond to regions where the most recent resurfacing due to impacts has occurred. Once again, the bright rayed crater Tycho stands out in this regard. The photo was made with the Ultraviolet Imaging Telescope flown aboard the Space Shuttle Endeavour in March 1995.
X-ray: The Moon, being a relatively peaceful and inactive celestial body, emits very little x-ray light, a form of radiation normally associated with highly energetic and explosive phenomena like black holes. This image was made by the orbiting ROSAT Observatory on June 29, 1990 and shows a bright hemisphere lit by oxygen, magnesium, aluminum and silicon atoms fluorescing in x-rays emitted by the Sun. The speckled sky records the “noise” of distant background X-ray sources, while the dark half of the Moon has a hint of illumination from Earth’s outermost atmosphere or geocorona that envelops the ROSAT observatory.
Gamma rays: Perhaps the most amazing image of all. If you could see the sky in gamma rays the Moon would be far brighter than the Sun as this dazzling image attempts to show. It was taken by the Energetic Gamma Ray Experiment Telescope (EGRET). High-energy particles (mostly protons) from deep space called cosmic rays constantly bombard the Moon’s surface, stimulating the atoms in its crust to emit gamma rays. These create a unique high-energy form of “moonglow”.
Astronomy in the 21st century is like having a complete piano keyboard on which to play compared to barely an octave a century ago. The Moon is more fascinating than ever for it.
Where Have All the Pulsars Gone? The Mystery at the Center of Our Galaxy
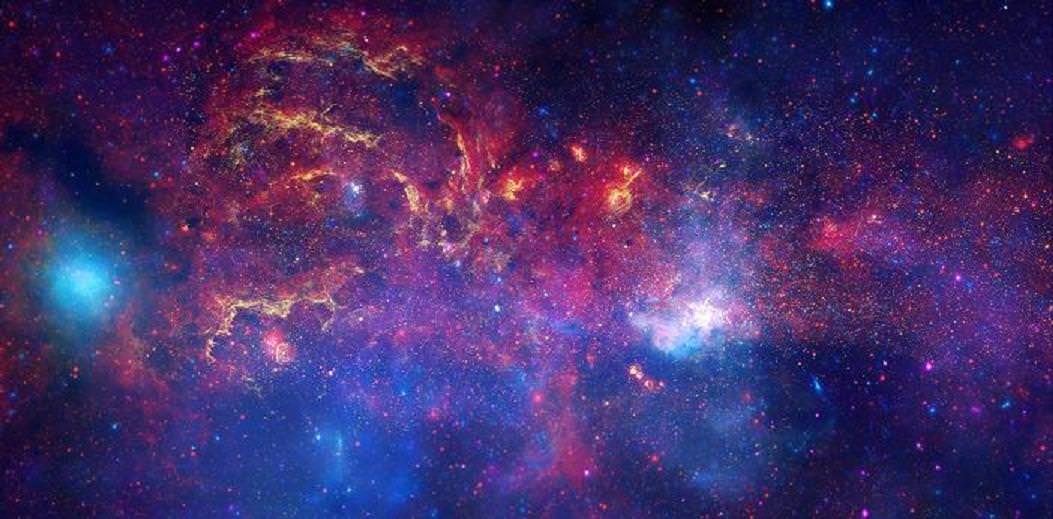
The galactic center is a happening place, with lots of gas, dust, stars, and surprising binary stars orbiting a supermassive black hole about three million times the size of our sun. With so many stars, astronomers estimate that there should be hundreds of dead ones. But to date, scientists have found only a single young pulsar at the galactic center where there should be as many as 50.
The question thus arises: where are all those rapidly spinning, dense stellar corpses known as pulsars? Joseph Bramante of Notre Dame University and astrophysicist Tim Linden of the University of Chicago have a possible solution to this missing-pulsar problem, which they describe in a paper accepted for publication in the journal Physical Review Letters.
Maybe those pulsars are absent because dark matter, which is plentiful in the galactic center, gloms onto the pulsars, accumulating until the pulsars become so dense they collapse into a black hole. Basically, they disappeared into the fabric of space and time by becoming so massive that they punched a hole right through it.
Dark matter, as you may know, is the theoretical mass that astrophysicists believe fills roughly a quarter of our universe. Alas, it is invisible and undetectable by conventional means, making its presence known only in how its gravitational pull interacts with other stellar objects.
One of the more popular candidates for dark matter is Weakly Interacting Massive Particles, otherwise known as WIMPs. Underground detectors are currently hunting for WIMPs and debate has raged over whether gamma rays streaming from the galactic center come from WIMPs annihilating one another.
In general, any particle and its antimatter partner will annihilate each other in a flurry of energy. But WIMPs don’t have an antimatter counterpart. Instead, they’re thought to be their own antiparticles, meaning that one WIMP can annihilate another.
But over the last few years, physicists have considered another class of dark matter called asymmetric dark matter. Unlike WIMPs, this type of dark matter does have an antimatter counterpart.
Asymmetric dark matter appeals to physicists because it’s intrinsically linked to the imbalance of matter and antimatter. Basically, there’s a lot more matter in the universe than antimatter – which is good considering anything less than an imbalance would lead to our annihilation. Likewise, according to the theory, there’s much more dark matter than anti-dark-matter.
Physicists think that in the beginning, the Big Bang should’ve created as much matter as antimatter, but something altered this balance. No one’s sure what this mechanism was, but it might have triggered an imbalance in dark matter as well – hence it is “asymmetric”.
Dark matter is concentrated at the galactic center, and if it’s asymmetric, then it could collect at the center of pulsars, pulled in by their extremely strong gravity. Eventually, the pulsar would accumulate so much mass from dark matter that it would collapse into a black hole.
The idea that dark matter can cause pulsars to implode isn’t new. But the new research is the first to apply this possibility to the missing-pulsar problem.
If the hypothesis is correct, then pulsars around the galactic center could only get so old before grabbing so much dark matter that they turn into black holes. Because the density of dark matter drops the farther you go from the center, the researchers predict that the maximum age of pulsars will increase with distance from the center. Observing this distinct pattern would be strong evidence that dark matter is not only causing pulsars to implode, but also that it’s asymmetric.
“The most exciting part about this is just from looking at pulsars, you can perhaps say what dark matter is made of,” Bramante said. Measuring this pattern would also help physicists narrow down the mass of the dark matter particle.
Credit: NASA, Caltech-JPL
But as Bramante admits, it won’t be easy to detect this signature. Astronomers will need to collect much more data about the galactic center’s pulsars by searching for radio signals, he claims. The hope is that as astronomers explore the galactic center with a wider range of radio frequencies, they will uncover more pulsars.
But of course, the idea that dark matter is behind the missing pulsar problem is still highly speculative, and the likelihood of it is being called into question.
“I think it’s unlikely—or at least it is too early to say anything definitive,” said Zurek, who was one of the first to revive the notion of asymmetric dark matter in 2009. The tricky part is being able to know for sure that any measurable pattern in the pulsar population is due to dark-matter-induced collapse and not something else.
Even if astronomers find this pulsar signature, it’s still far from being definitive evidence for asymmetric dark matter. As Kathryn Zurek of the Lawrence Berkeley National Laboratory explained: “Realistically, when dark matter is detected, we are going to need multiple, complementary probes to begin to be convinced that we have a handle on the theory of dark matter.”
And asymmetric dark matter may not have anything to do with the missing pulsar problem at all. The problem is relatively new, so astronomers may find more plausible, conventional explanations.
“I’d say give them some time and maybe they come up with some competing explanation that’s more fleshed out,” Bramante said.
Nevertheless, the idea is worth pursuing, says Haibo Yu of the University of California, Riverside. If anything, this analysis is a good example of how scientists can understand dark matter by exploring how it may influence astrophysical objects. “This tells us there are ways to explore dark matter that we’ve never thought of before,” he said. “We should have an open mind to see all possible effects that dark matter can have.”
There’s one other way to determine if dark matter can cause pulsars to implode: To catch them in the act. No one knows what a collapsing pulsar might look like. It might even blow up.
“While the idea of an explosion is really fun to think about, what would be even cooler is if it didn’t explode when it collapsed,” Bramante said. A pulsar emits a powerful beam of radiation, and as it spins, it appears to blink like a lighthouse with a frequency as high as several hundred times per second. As it implodes into a black hole, its gravity gets stronger, increasingly warping the surrounding space and time.
Studying this scenario would be a great way to test Einstein’s theory of general relativity, Bramante says. According to theory, the pulse rate would get slower and slower until the time between pulses becomes infinitely long. At that point, the pulses would stop entirely and the pulsar would be no more.
Further Reading: APS Physics, WIRED
Radio Telescopes Help Astronomers Tune In To Nova Generated Gamma Rays
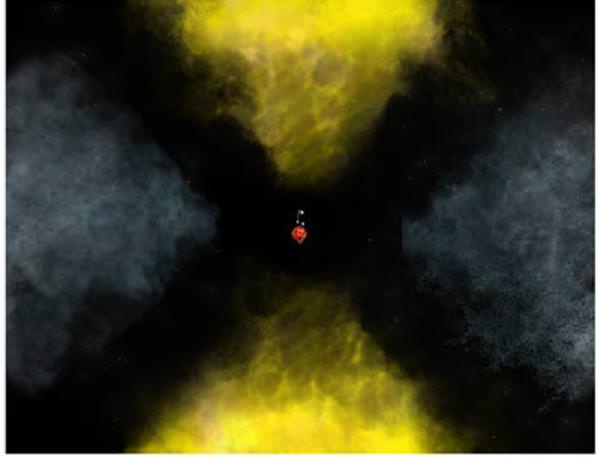
Over two years ago, the Fermi-LAT Collaboration observed an “ear and eye opening” event – the exact location where a stellar explosion called a nova emitted one of the most energentic forms of electromagnetic waves… gamma rays. When it was first detected in 2012, it was something of a mystery, but the findings could very well point to what may cause gamma ray emissions.
“We not only found where the gamma rays came from, but also got a look at a previously-unseen scenario that may be common in other nova explosions,” said Laura Chomiuk, of Michigan State University.
A nova? According to the Fermi researchers, a classical nova results from runaway thermonuclear explosions on the surface of a white dwarf that accretes matter from a low-mass main-sequence stellar companion. As it gathers in material, the thermonuclear event expels debris into surrounding space. However, astronomers didn’t expect this “normal” event to produce high energy gamma rays!
Explains the Fermi-LAT team: “The gamma-ray detections point to unexpected high-energy particle acceleration processes linked to the mass ejection from thermonuclear explosions in an unanticipated class of Galactic gamma-ray sources.”
While NASA’s Fermi spacecraft was busy watching a nova called V959 Mon, some 6500 light-years from Earth, other radio telescopes were also busy picking up on the gamma ray incidences. The Karl G. Jansky Very Large Array (VLA) was documenting radio waves coming from the nova. The source of these emissions could be subatomic particles moving at nearly the speed of light interacting with magnetic fields – a condition needed to help produce gamma rays. These findings were backed up by the extremely-sharp radio “vision” of the Very Long Baseline Array (VLBA) and the European VLBI network. They revealed two knots in the radio observations – knots which were moving away from each other. Additional studies were done with e-MERLIN in the UK, and another round of VLA observations in 2014. Now astronomers could begin to piece together the puzzle of how radio knots and gamma rays are produced.
According to the NRAO news release, the white dwarf and its companion give up some of their orbital energy to boost some of the explosion material, making the ejected material move outward faster in the plane of their orbit. Later, the white dwarf blows off a faster wind of particles moving mostly outward along the poles of the orbital plane. When the faster-moving polar flow hits the slower-moving material, the shock accelerates particles to the speeds needed to produce the gamma rays, and the knots of radio emission.
“By watching this system over time and seeing how the pattern of radio emission changed, then tracing the movements of the knots, we saw the exact behavior expected from this scenario,” Chomiuk said.
But the V959 Mon observations weren’t the end of the story. According to Fermi-LAT records, in 2012 and 2013, three novae were detected in gamma rays and stood in contrast to the first gamma-ray detected nova V407 Cygni 2010, which belongs to a rare class of symbiotic binary systems. Despite likely differences in the compositions and masses of their white dwarf progenitors, the three classical novae are similarly characterized as soft spectrum transient gamma-ray sources detected over 2-3 week durations.
“This mechanism may be common to such systems. The reason the gamma rays were first seen in V959 Mon is because it’s close,” Chomiuk said. Because the type of ejection seen in V959 Mon also is seen in other binary-star systems, the new insights may help astronomers understand how those systems develop. This “common envelope” phase occurs in all close binary stars, and is poorly understood. “We may be able to use novae as a ‘testbed’ for improving our understanding of this critical stage of binary evolution,” explains Chomiuk.
Original Story Source: Radio Telescopes Unravel Mystery of Nova Gamma Rays from National Radio Astronomy Observatory. Chomiuk worked with an international team of astronomers. The researchers reported their findings in the scientific journal “Nature”.
Surprise! Classical Novae Produce Gamma Rays
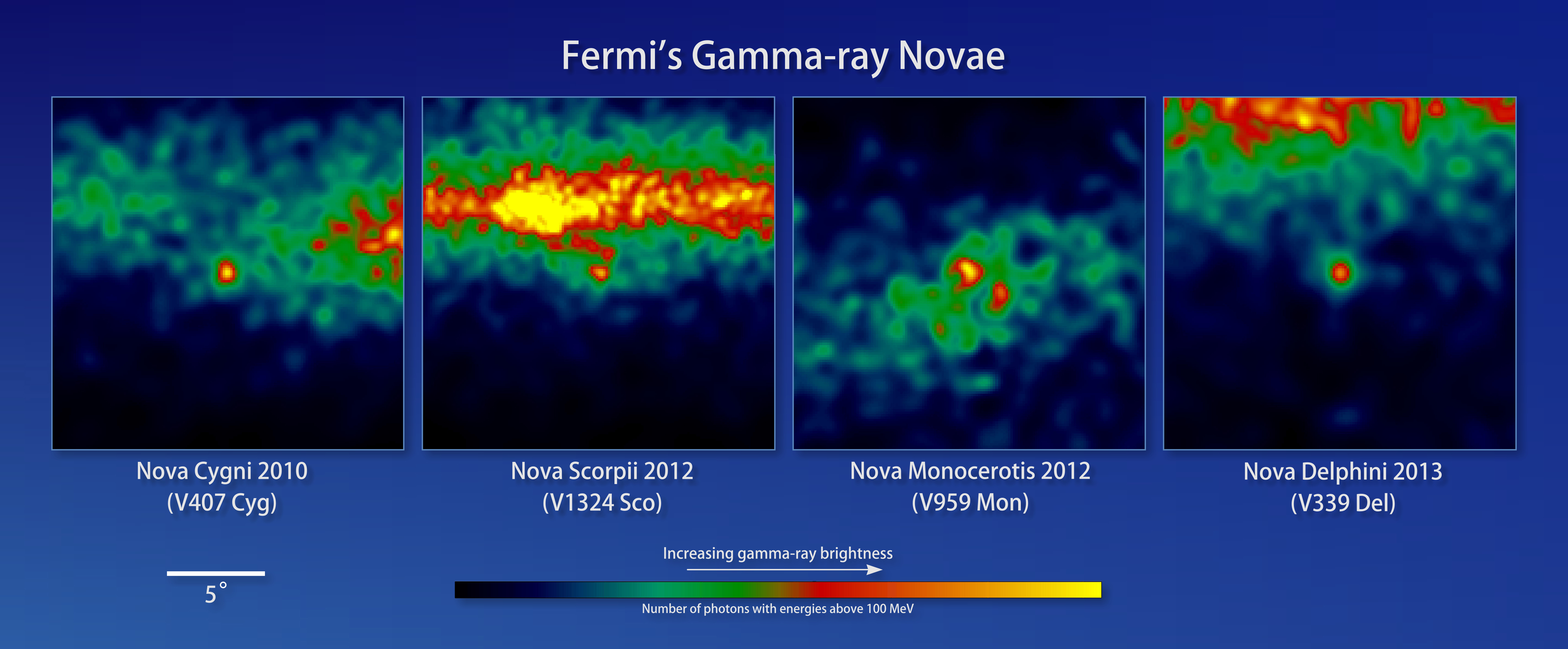
In a classical nova, a white dwarf siphons material off a companion star, building up a layer on its surface until the temperature and pressure are so high (a process which can take tens of thousands of years) that its hydrogen begins to undergo nuclear fusion, triggering a runaway reaction that detonates the accumulated gas.
The bright outburst, which releases up to 100,000 times the annual energy output of our Sun, can blaze for months. All the while, the white dwarf remains intact, with the potential of going nova again.
It’s a relatively straightforward picture — as far as complex astrophysics goes. But new observations with NASA’s Fermi Gamma-ray Space Telescope unexpectedly show that three classical novae — V959 Monocerotis 2012, V1324 Scorpii 2012, and V339 Delphini 2013 — and one rare nova, also produce gamma rays, the most energetic form of light.
“There’s a saying that one is a fluke, two is a coincidence, and three is a class, and we’re now at four novae and counting with Fermi,” said lead author Teddy Cheung from the Naval Research Laboratory in a press release.
The first nova detected in gamma rays was V407 Cygni — a rare star system in which a white dwarf interacts with a red giant — in March 2010.
One explanation for the gamma-ray emission is that the blast from the nova hits the hefty wind from the red giant, creating a shock wave that accelerates any charged particles to near the speed of light. These rapid particles, in turn, produce gamma rays.
But the gamma-ray peak follows the optical peak by a couple of days. This likely happens because the material the white dwarf ejects initially blocks the high-energy photons from escaping. So the gamma rays cannot escape until the material expands and thins.
But the later three novae are from systems that don’t have red giants and therefore their winds. There’s nothing for the blast wave to crash into.
“We initially thought of V407 Cygni as a special case because the red giant’s atmosphere is essentially leaking into space, producing a gaseous environment that interacts with the explosion’s blast wave,” said coauthor Steven Shore from the University of Pisa. “But this can’t explain more recent Fermi detections because none of those systems possess red giants.”
In a more typical system it’s likely that the blast creates multiple shock waves that expand into space at slightly different speeds. Faster shocks could blast into slower ones, creating the interaction necessary to produce gamma rays. Although, the team remains unsure if this is the case.
Astronomers estimate that between 20 and 50 novae occur each year in the Milky Way galaxy. Most go undetected, their visible light obscured by intervening dust, and their gamma rays dimmed by distance. Hopefully, future observations of nearby novae will shed light on the mysterious process producing gamma rays.
The results will appear in Science on August 1.