It may seem all but impossible to determine how the Solar System formed, given that it happened roughly 4.5 billion years ago. Luckily, much of the debris that was left over from the formation process is still available today for study, circling our Solar System in the form of rocks and debris that sometimes make their way to Earth.
Among the most useful pieces of debris are the oldest and least altered type of meteorites, which are known as chondrites. They are built mostly of small stony grains, called chondrules, that are barely a millimeter in diameter.
And now, scientists are being provided with important clues as to how the early Solar System evolved, thanks to new research based on the the most accurate laboratory measurements ever made of the magnetic fields trapped within these tiny grains.
To break it down, chondrite meteorites are pieces of asteroids — broken off by collisions — that have remained relatively unmodified since they formed during the birth of the Solar System. The chondrules they contain were formed when patches of solar nebula – dust clouds that surround young suns – was heated above the melting point of rock for hours or even days.
The dust caught in these “melting events” was melted down into droplets of molten rock, which then cooled and crystallized into chondrules. As chondrules cooled, iron-bearing minerals within them became magnetized by the local magnetic field in the gas cloud. These magnetic fields are preserved in the chondrules right on up to the present day.

The chondrule grains whose magnetic fields were mapped in the new study came from a meteorite named Semarkona – named after the town in India where it fell in 1940.
Roger Fu of MIT – working under Benjamin Weiss – was the chief author of the study; with Steve Desch of Arizona State University’s School of Earth and Space Exploration attached as co-author.
According to the study, which was published this week in Science, the measurements they collected point to shock waves traveling through the cloud of dusty gas around the newborn sun as a major factor in solar system formation.
“The measurements made by Fu and Weiss are astounding and unprecedented,” says Steve Desch. “Not only have they measured tiny magnetic fields thousands of times weaker than a compass feels, they have mapped the magnetic fields’ variation recorded by the meteorite, millimeter by millimeter.”
The scientists focused specifically on the embedded magnetic fields captured by “dusty” olivine grains that contain abundant iron-bearing minerals. These had a magnetic field of about 54 microtesla, similar to the magnetic field at Earth’s surface (which ranges from 25 to 65 microtesla).
Coincidentally, many previous measurements of meteorites also implied similar field strengths. But it is now understood that those measurements detected magnetic minerals that were contaminated by the Earth’s own magnetic field, or even from the hand magnets used by the meteorite collectors.
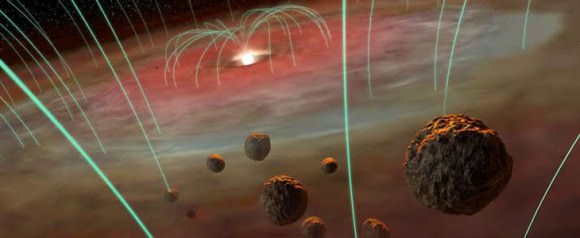
Credit: Hernán Cañellas
“The new experiments,” Desch says, “probe magnetic minerals in chondrules never measured before. They also show that each chondrule is magnetized like a little bar magnet, but with ‘north’ pointing in random directions.”
This shows, he says, that they became magnetized before they were built into the meteorite, and not while sitting on Earth’s surface. This observation, combined with the presence of shock waves during early solar formation, paints an interesting picture of the early history of our Solar System.
“My modeling for the heating events shows that shock waves passing through the solar nebula is what melted most chondrules,” Desch explains. Depending on the strength and size of the shock wave, the background magnetic field could be amplified by up to 30 times. “Given the measured magnetic field strength of about 54 microtesla,” he added, “this shows the background field in the nebula was probably in the range of 5 to 50 microtesla.”
There are other ideas for how chondrules might have formed, some involving magnetic flares above the solar nebula, or passage through the sun’s magnetic field. But those mechanisms require stronger magnetic fields than what has been measured in the Semarkona samples.
This reinforces the idea that shocks melted the chondrules in the solar nebula at about the location of today’s asteroid belt, which lies some two to four times farther from the sun than the Earth’s orbits.
Desch says, “This is the first really accurate and reliable measurement of the magnetic field in the gas from which our planets formed.”
Further Reading: ASU